Air Cooled Chillers
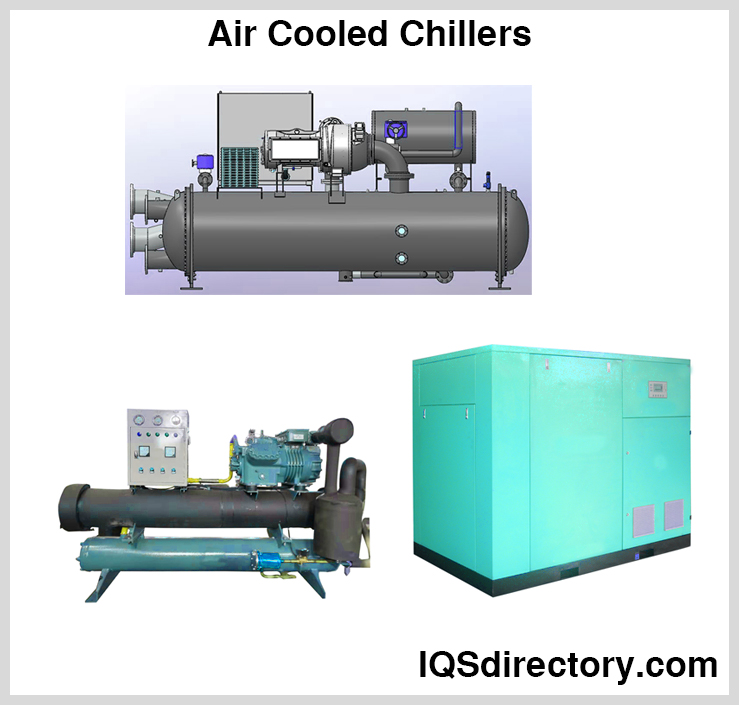
Air cooled chillers are refrigeration systems that cool fluids and work in tandem with the air handler system of a facility. Air cooled chillers are types of chillers that rely on the use of fans to reject heat outside the...
Please fill out the following form to submit a Request for Quote to any of the following companies listed on Laser Chillers .
This article will take an in-depth look at laser coolers and laser cooling.
The article will bring more detail on topics such as:
This chapter delves into the basics of laser cooling, explores different cooling techniques, and explains how laser coolers function.
Laser cooling encompasses various strategies designed to decrease the temperature of atomic and molecular samples close to absolute zero. These techniques take advantage of the phenomenon where an atom or molecule changes its momentum upon re-emitting a photon, leading to a decline in its overall kinetic energy.
In a particle collection, the thermodynamic temperature correlates with the distribution of particle velocities. Thus, a more uniform velocity distribution among particles implies a lower temperature.
Laser cooling methods leverage atomic spectroscopy alongside the mechanical effects of light to achieve a narrower velocity distribution in particle groups, thereby cooling them.
Doppler cooling stands out among the various techniques employed in laser cooling; its practicality often leads to it being equated with laser cooling itself.
Other laser cooling techniques include Sisyphus cooling, resolved sideband cooling, Raman sideband cooling, velocity selective coherent population trapping (VSCPT), gray molasses, cavity-mediated cooling, polarization gradient cooling, anti-Stokes cooling in solids, electromagnetically induced transparency (EIT) cooling, and the utilization of the Zeeman slower.
Doppler cooling is used to trap and reduce atom motion, effectively cooling substances. A stationary atom does not experience a shift in laser light and therefore does not absorb the photon.
An atom moving away perceives the light as red-shifted and does not absorb the photon. However, an atom moving towards the laser finds the light blue-shifted and absorbs it, slowing down. The absorption excites the atom, elevating an electron to a higher state, and upon re-emission of a photon, which occurs in a random direction, there is no net momentum change over numerous emissions.
The technique's core principle is that photons interacting with an atom are mainly unaffected by it. The atom is transparent to most photon frequencies, resonating only at very narrow frequency bands. Interactions occur at specific colors, unlike the mixed colors of white light.
When a resonant photon engages with the atom, it's briefly absorbed. The atom re-emits the photon in random directions, which does not significantly affect the thermal energy of atoms, contrary to the common belief about lasers increasing thermal energy.
If nearly motionless and carefully controlled to frequency, an atom remains unresponsive to most laser frequencies, reacting only at certain electromagnetic frequencies. When matched with these frequencies, the atom absorbs a photon, gains its momentum, and moves with the photon. After a short while, the atom emits a photon, typically in a random direction, potentially losing gained momentum. If the re-emitted photon travels oppositely, more momentum is acquired in that direction.
To maintain momentum, this causes the atom's velocity to double. Often, re-emitted photons impart a sideways thrust. Laser frequency is adjusted by using monochromatic light, slightly below a resonant frequency. Initially, frequency well under the resonant frequency passes through. Eventually, moving atoms absorb photons and slow, while atoms moving away remain unaffected.
On a velocity graph, faster right-moving atoms are dots on the right, and left-moving ones are dots on the left. A narrow band on the left represents velocities absorbing photons from the left laser. Within this band, each collision slows atoms based on the photon’s momentum. Adjusting the laser frequency narrows the interaction band, causing a velocity shift towards zero, achieving the cold state.
Sisyphus cooling achieves temperatures beneath the Doppler cooling limit by employing two counter-propagating laser beams with orthogonal polarizations. When atoms navigate the potential landscape generated by these standing wave lasers, they lose kinetic energy. Optical pumping transitions them to lower energy states, reducing total atomic energy.
The principle involves polarization gradients created by counter-propagating lasers with orthogonal polarizations that lead to a standing wave. This gradient alternates between circularly polarized light, varying over λ/2, and mirrors about the y-z plane. Depending on position, these beams can create a π/2 phase difference. Polarization frees into circular when there's no phase difference, transitioning to linear. In these gradient areas, ellipticity appears.
This method cools tightly bound atoms and ions beyond the Doppler limit, approaching ground states. It's mainly applied to atoms intensely trapped, reaching the lowest motion energy level.
Cold and trapped, atoms resemble quantum mechanical harmonic oscillators. If spontaneous decay rates are lower than atom vibrational frequencies, these systems divide into internal levels, each with vibrational states.
Effective laser cooling requires aligning laser frequency to the red sideband. Spontaneous emissions occur at the carrier frequency, cooling the ion by one vibrational level when recoil energy is negligible to vibrational quantum energy. Efficient resolved sideband cooling begins at low initial temperatures.
Typically, particles first reach the Doppler limit, then undergo several sideband cycles. Subsequently, measurements or state manipulations occur. The narrow quadrupole transition for cooling connects ground states to a long-lived state, essential for cooling efficiency.
Raman cooling, an atomic physics technique, achieves temperatures beneath Doppler cooling limits using optical methods. This surpasses Doppler cooling's constraints by employing optical molasses or superimposed optical lattices for heightened atomic temperature control.
Raman cooling uses twin lasers to transition atoms between hyperfine states. The first excites the atom below the actual transition frequency to a virtual state, while the second de-excites it back. The beams align their frequency differences to the transition between hyperfine states, enabling effective cooling.
Raman sideband cooling starts with atoms in a magneto-optical trap, intensifying an optical lattice to trap a significant atom fraction. Each lattice site is akin to a harmonic trap if lattice lasers have ample power. Initially, atoms are in an excited harmonic level, but the goal is transitioning to the ground state at each site.
For a two-level atom (F=1), threefold degenerate m-states (m=-1, 0, 1) require degeneracy lifting via magnetic fields and Zeeman splitting. Zeeman splitting must align with energy spacing between harmonic potential levels.
Raman processes allow transitions to states with decreased magnetic moments and vibrational states. Post-transfer, atoms in the lowest lattice vibrational state, pumped optically to the m=1 state, do not further change vibrationally if temperature is low compared to pump frequency, facilitating cooling. Adjust laser power and timing for effective vibrational state transfer.
Cooling enables higher atom densities at low temperatures. Although photon recoil complicates the process, operating in the Lamb-Dicke regime mitigates it.
Gray molasses, a sub-Doppler laser cooling method, merges Sisyphus cooling with a dark state, untouched by resonant lasers, for experiments in ultra-cold atomic physics with poor-resolved species, like lithium and potassium isotopes. It achieves temperatures under the Doppler limit.
Unlike magneto-optical traps, gray molasses, limited to atom slowing but not trapping, efficiently cools but operates only for milliseconds before trapping and extra cooling. It relies on two-photon and Raman transitions between hyperfine-split ground states, mediated through excitation.
Bright and dark states, ground state orthogonal superpositions, interact via dipole-driven transitions. Bright states lead to excited states; dark states, accessible from excited states through spontaneous emission, oscillate due to fluctuating momentum-dependent state coupling.
The polarization gradient creates a sinusoidal bright state-potential. Atoms lose kinetic energy towards potential maxima, corresponding to electric dipole-transition enabling circular polarizations. Atoms transition optically pumped back to dark state-excited cycles, creating Sisyphus-like cooling. Cold atoms enter and exit cycles through bright, excited to dark states.
Through coherent optical nonlinearity, a medium becomes transparent over a narrow spectral range centered on an absorption line, allowing "slow light." It involves quantum interference permitting light passage through otherwise opaque mediums and utilises two highly coherent optical fields interacting with three distinct quantum states.
Configuring the probe field close to resonance reveals transparency and significant dispersion. The principle relies on atomic state transition probability amplitude interference. Optimizing cooling requires three atom-level systems: ground, excited, and intermediate states.
Ensuring dipole coupling between states, coupling lasers excite states, while weak cooling lasers induce transitions. Transparency cooling ensues when ground-stable state differences equate carrier transition energies. Alignment to Fano-like feature-dark resonance enhances cooling overother methods through larger excitation probability ratios.
Developed to explain cooling below Doppler limitations, polarization gradient cooling achieves microkelvin to sub-microkelvin temperatures. It generates spatially varying gradients through superposed orthogonal polarization light beams, creating varying polarization states.
Orthogonal linear polarizations oscillate between linear and circular over half-wavelength spatial periods, while circular ones rotate linearly along the propagation axis, yielding similar cooling yet differing mechanisms.
In linear polarizations, cooling arises from periodic atomic ground state shifts leading to Sisyphus processes. Circular ones engender Zeeman level imbalances due to motion-induced polarization differences, impacting radiation-induced atomic motion resistance, reaching recoil limits. However, its narrow capture range necessitates prior Doppler cooling despite enabling lower temperatures.
Implemented using a 3D optical setup, it involves six lasers perpendicular to an atomic ensemble, each counterbalanced, generating orthogonal light polarization. Laser frequency detunes from ground-excited state transitions. To avoid irrelevant state transitions, a re-pumping laser returns atoms to ground states.
Reduction and detuning follow Doppler cooling. Using the time-of-flight method, laser shutdown permits ensemble expansions measured by probe beams after delays to track expansion rates, indicative of atomic temperature.
This chapter explores the most common laser cooling devices and the various types of chillers used in laser system temperature control. By understanding the range of instruments and chiller architectures available, users can improve laser performance, extend equipment life, and maintain essential temperature stability in applications ranging from industrial laser cutting to analytical laboratories.
Laser cooling technology relies on several advanced instruments designed for precision temperature management and particle manipulation. Key laser cooling devices include:
The Zeeman slower is a fundamental tool in quantum optics and atomic physics research. Used to reduce the kinetic energy and temperature of an atomic beam from room temperature to just a few Kelvin, it creates optimal conditions for trapping atoms in applications such as quantum computing, atomic clocks, and Bose-Einstein condensate experiments. Atoms typically start with velocities of several hundred meters per second; as they progress through the slower, their velocities are reduced dramatically, enabling higher-precision control.
This instrument operates on Doppler laser cooling principles by tuning a laser to the resonance frequency of a two-level atom. As atoms travel along a defined path, they absorb photons from a counter-propagating laser beam, which imparts a recoil momentum—slowing the atom according to conservation of momentum. Following absorption, atoms return to their ground state through spontaneous emission, which redistributes energy via randomly directed photons.
This process results in overall cooling along the axis of the laser, making Zeeman slowers essential for high-precision laser manipulation and spectroscopy. Their precise operation enhances the performance and accuracy of cold atom traps, optical lattices, and advanced measurement instruments.
The ion pump is a high-efficiency vacuum pump widely used within atomic physics, particle accelerators, and laser cooling systems. It operates by sputtering a metal surface to create ultra-high vacuum (UHV) environments, crucial for stable laser cooling experiments and low contamination levels. Ion pumps can reach pressures as low as 10-11 mbar, supporting both basic and advanced vacuum equipment needs.
The process involves ionizing residual gas molecules within the chamber and then accelerating these ions toward a cathode under a high voltage (3–7 kV). This high-voltage process enables ion pumps to trap and chemically react with gas molecules, permanently removing them from the chamber and ensuring a highly controlled, contaminant-free environment.
Chiller systems are critical for laser cooling applications, where heat must be managed to ensure consistent laser output, minimal thermal drift, and prolonged component life. Chillers are categorized according to their cooling mechanisms, heat rejection methods, and compressor or absorption types, each suited for specific laser power, throughput, and integration requirements.
The centrifugal chiller uses centrifugal compression to convert kinetic energy into static pressure, effectively increasing both the temperature and pressure of the refrigerant. With impeller blades efficiently pulling and compressing the refrigerant, this chiller design is ideal for high-capacity, centralized cooling systems common in laser fabrication facilities, semiconductor manufacturing, and industrial-scale laser machining. Centrifugal chillers offer significant energy efficiency and reliability, with low maintenance requirements and the ability to handle large thermal loads.
Laser chiller systems are engineered to maintain precise temperature regulation for all types of laser equipment, including fiber lasers, CO2 lasers, Nd:YAG lasers, excimer lasers, and diode lasers. These chillers ensure optimal laser output power, reduce the risk of wavelength drift, and protect sensitive optical and electronic components from overheating.
Laser chillers feature advanced cooling technologies such as precision temperature controllers, recirculating water systems, and automatic safety cutoffs. Digital monitoring and remote diagnostics are increasingly common for remote or unattended installations. In demanding research and medical applications, dual-zone chillers allow for individual temperature control of separate laser system sections, maximizing flexibility.
Applications such as CO2 lasers, excimer lasers, and high-power industrial laser exciters demand reliable and efficient chiller water-cooling systems to ensure stable performance, minimize downtime, and extend equipment service life.
Choosing a Laser Chiller: When selecting a laser chiller, consider laser type, cooling capacity, temperature stability (often ±0.1°C), flow rate, and integration options with broader facility cooling systems. Selecting from specialized manufacturers or custom chiller suppliers can improve fit and maximize operational efficiency.
Absorption chillers provide an alternative cooling technology that uses a heat-driven generator—powered by steam or hot water—to evaporate the refrigerant. This solution is often used in facilities prioritizing energy efficiency and sustainability, such as laboratories, hospitals, or locations with waste heat recovery systems. Absorption chillers have minimal moving parts, resulting in low noise and reduced vibration, which can be advantageous for sensitive laser installations.
Within the unit, the refrigerant vapor is condensed and absorbed into a solution, where heat is released. The resulting liquid mixture is pumped back to the generator for repeat cycling. These systems are ideal for large facilities looking to optimize operational cost and leverage existing energy infrastructure.
Air cooled chillers extract heat from water (or glycol-based coolants) and dissipate it directly into the surrounding air, making them ideal for environments without easy access to water infrastructure or where discharged heat is not a concern. These chillers are popular with small-to-midsize industrial lasers, laboratory lasers, and medical laser systems due to lower installation costs and easier maintenance.
The evaporator inside the chiller absorbs heat from the process water, while the condenser coil and built-in fans discharge this heat to the air. Air cooled chillers are modular, scalable, and often installed outdoors, providing added flexibility for operational layouts. Suitable for environments where water conservation is a priority, these systems reduce facility utility costs while reliably protecting sensitive laser equipment from thermal fluctuations.
Additional Types of Laser Cooling Solutions: Besides traditional chillers, emerging laser cooling applications use direct-to-chip liquid cooling, closed-loop chillers for laboratory lasers, and hybrid systems combining air and water cooling. Each method offers specific advantages based on space constraints, noise levels, temperature accuracy, and required cooling power.
This chapter explores the diverse applications and cutting-edge uses of laser coolers and the advanced process of laser cooling technology across scientific research, industrial manufacturing, and emerging quantum technologies.
Laser cooling techniques are essential in high-resolution spectroscopic measurements, including frequency standards in optical atomic clocks. These ultra-stable atomic clocks depend on extremely cold atoms or ions, which dramatically reduce Doppler broadening effects and achieve exceptional timekeeping precision. By suppressing atomic motion, laser cooling enables more accurate atomic transitions and supports advancements in time metrology and synchronization systems used in global navigation and telecommunications.
Additionally, laser cooling plays a critical role in ultra-precise gravitational field measurements. These measurements are crucial not only for gravitational physics research but also for practical applications such as oil and mineral exploration. Advanced gravimeters, relying on laser-cooled atoms and exploiting Doppler shifts during free fall or through Bloch oscillations, deliver unprecedented sensitivity when detecting tiny variations in the Earth’s gravitational field. This has revolutionized the accuracy of subsurface mapping and geophysical surveys.
Laser cooling technologies are indispensable in precision lithography, where they enable the atomically precise fabrication of semiconductor structures and nanomaterials. Utilizing cold atomic beams allows for controlled, high-accuracy patterning at the nanoscale, which is foundational to microelectronics manufacturing and photonics device production. Moreover, laser coolers are at the heart of quantum physics experiments near absolute zero temperature, where unique quantum phenomena—such as Bose-Einstein condensation—can be achieved and studied. These ultra-cold atomic gases pave the way for advancements in quantum simulation, quantum computation, and fundamental tests of quantum mechanics.
Furthermore, laser cooling is integral to the development of vibration-free optical refrigeration systems and radiation-balanced solid-state lasers. By precisely managing thermal loads in laser amplifiers and sensitive photonic components, these advanced cooling solutions increase operational efficiency and extend the performance envelope of high-power laser systems used in medical devices, aerospace instrumentation, and industrial laser processing.
Other emerging applications of laser coolers include advancements in quantum sensing, where cooled ions and atoms yield ultra-high sensitivity for detecting magnetic and electric fields, as well as in atomic interferometry, which underpins next-generation inertial navigation systems. As the need for highly controlled thermal environments grows in semiconductor manufacturing, laboratory research, and photonic device fabrication, laser coolers and laser cooling technology continue to drive innovation, enabling breakthroughs in both fundamental science and cutting-edge industry solutions.
The word laser stands for Light Amplification by Stimulated Emission of Radiation, which occurs when electrons in optical materials like glass, crystals, or gases absorb energy from an electrical current. This energy causes the electrons to become excited, moving from a low energy state to a higher one, thus producing electromagnetic radiation.
In regular light, wavelengths vary in direction and length, with each wavelength representing a different color, resulting in what appears to the naked eye as white light. Conversely, a laser beam consists of coherent light, where all the wavelengths align and travel in the same direction, with their peaks and valleys synchronized. This focused light generates substantial heat energy.
When a laser beam is directed onto a surface, its light energy transforms into heat, which can cut, melt, or burn the material. The heat generated must be dissipated to maintain the laser's efficiency and prevent damage. Industrial lasers use various cooling methods, including chillers, to maintain a consistent temperature.
Laser chillers are specifically designed to remove excess heat from the laser process, helping to maintain the laser's wavelength stability and ensuring the beam quality. High-powered lasers typically require water-cooled chillers, while lower-powered lasers may be sufficiently cooled with fans or other cooling mechanisms.
When selecting a laser chiller, it's crucial to choose one that is compatible with the specific type of laser in use. Laser chillers are commonly used with:
Cold plates play a crucial role in laser cooling systems and are typically used in conjunction with recirculating chillers. They come in various designs, including tubed cold plates and aluminum vacuum-brazed versions.
Cold plates are often installed on lasers to receive cooling fluids supplied by a chiller. The heated liquid from the cooling process is then routed back to the chiller. Additionally, in some designs, the cold plate may function as the electrodes within the laser system.
Thermoelectric coolers (TECs) operate based on the Peltier effect, which involves generating a heat flux at the junction of two different materials when a direct current (DC) is applied. In a TEC, positive and negative semiconductors are arranged parallel in the thermal pathway and in series in the electrical pathway. When a voltage is applied, electrons transport heat from one side to the other, cooling one side while heating the opposite side. Fans then dissipate the accumulated heat into the surrounding air.
A TEC assembly can be directly attached to a cold plate or used to cool a refrigerant liquid. Typically, TECs are suited for applications requiring cooling capacities of up to 400 watts.
Vapor compression chillers operate by circulating a refrigerant through a sequence of components: an evaporator, compressor, condenser, and expansion valve. This method is effective for cooling high power loads while consuming relatively little energy. In laser systems, vapor compression chillers can be adapted by adjusting the evaporator cycle, whereas other methods might involve running the refrigerant directly through the cold plate.
The compressor-based vapor liquid chiller is a widely utilized cooling technique for high-powered lasers. These chillers are capable of managing cooling loads up to 10 kW and are compatible with various types of lasers.
The miniature rotary compression system is now a popular choice for laser cooling applications. Advances in rotary compressor technology have enabled the creation of compact systems capable of handling heat loads up to 100 watts. This smaller, more efficient design has become widely adopted for various laser cooling needs.
Miniature rotary compressor chillers offer portability, precise temperature control, and a cooling capacity ranging from 3 kW to 140 kW. They operate effectively within a temperature range of 20°F to 80°F.
In a direct expansion chiller system, refrigerant circulates directly through the cold plate, driven by the compressor. This approach eliminates the necessity for a separate water cooling loop, simplifying the overall system design. By removing the need for an additional coolant loop, this method provides precise temperature control through the isothermal phase change of the refrigerant as it passes over the cold plate.
Direct expansion systems have the typical components of a compressor, condenser, expansion valve, and evaporator, which absorbs the heat directly. They are a compact cooling solution with a miniature rotary compressor. The advantage of the system is the precision at which it controls the temperature. Direct expansion cooling systems are designed for high heat flux applications such as laser cutting and burning.
In general, laser cooling refers to the use of dissipative light forces so as to reduce the random motion of contained atoms and also reduce the temperature of small particles like atoms and ions. The temperature attained can result in different magnitudes all depending on the type of mechanism used.
Air cooled chillers are refrigeration systems that cool fluids and work in tandem with the air handler system of a facility. Air cooled chillers are types of chillers that rely on the use of fans to reject heat outside the...
A chiller is a cooling mechanism designed to produce fluids that can lower temperatures by removing heat from the fluid. The type and use of a chiller depends on the required temperature and kind of refrigerant, which can be a liquid or a gas...
A glycol chiller is a chilling system that uses a percentage of glycol mixed with water to create extremely low temperatures far beyond the freezing point of water. The two types of glycol are ethylene glycol based or propylene glycol based...
A water chiller, or chilled water system, is a type of refrigeration system which uses water as a secondary refrigerant. They are used for larger, more complex, heating, ventilating, air conditioning, and refrigeration (HVACR) applications...
Cooling towers are specialized heat exchangers that remove heat from water mainly by means of latent heat loss from evaporation while coming into contact with an airstream. Aside from evaporative cooling, water is also cooled by sensible heat transfer due to the temperature difference between air and water...
Heat exchangers are pieces of equipment used to transfer heat between two or more fluids. This process usually involves abundant working or utility media such as water or air that rejects or absorbs heat from a more valuable fluid such as crude oil, petrochemical feedstocks, and fluidized products...
Open loop cooling tower makes use of direct contact with the air in order to cool down the water. It is essentially a heat exchanger. In these types of cooling towers, there is the partial heat transfer due to heat exchange between...
A plate heat exchanger (PHE) is a compact type of heat exchanger that utilizes a series of thin metal plates to transfer heat from one fluid to the other. These fluids are typically at different temperatures...
A shell and tube heat exchanger (STHE) is a type of heat exchanging device constructed using a large cylindrical enclosure, or shell, that has bundles of perfectly spaced tubing compacted in its interior. Heat exchanging is the transfer of heat from one substance or medium to a similar substance or medium...